Automated Surface Subdivision and Tool Path Generation for 3+2-Axis CNC Machining of Sculptured Parts
Computer Numerical Control (CNC) machining is a cornerstone of modern manufacturing, enabling the precise fabrication of complex components across industries such as aerospace, automotive, and medical device production. Among the various CNC techniques, 3+2-axis CNC machining, also referred to as indexed 5-axis machining, has emerged as a cost-effective and versatile method for producing sculptured parts—components characterized by freeform, non-planar surfaces. Unlike continuous 5-axis machining, which synchronizes five axes simultaneously, 3+2-axis machining combines the simplicity of 3-axis tool paths with two additional discrete rotational axes, typically provided by an indexing or rotary table. This approach enhances the ability of standard 3-axis CNC machines to tackle complex geometries while maintaining high accuracy and reducing costs compared to full 5-axis systems.
A critical challenge in 3+2-axis CNC machining of sculptured parts lies in generating efficient tool paths that ensure precision, minimize machining time, and avoid tool interference. Automated surface subdivision and tool path generation techniques address this challenge by dividing complex surfaces into manageable patches and optimizing tool paths for each patch. These methods leverage computational algorithms to analyze surface geometry, determine optimal machining setups, and generate interference-free tool paths, significantly improving productivity and part quality. This article provides a comprehensive exploration of automated surface subdivision and tool path generation for 3+2-axis CNC machining of sculptured parts, covering theoretical foundations, methodologies, applications, and future research directions.
Historical Context and Evolution of CNC Machining
The development of CNC machining began in the mid-20th century with the advent of numerical control systems, which automated machine tool operations using punched tape. Early CNC machines operated primarily in 2 or 3 axes, limiting their ability to handle complex geometries. The introduction of 5-axis CNC machines in the 1980s marked a significant advancement, enabling continuous control of tool orientation and position. However, the high cost and complexity of 5-axis systems prompted the development of 3+2-axis machining as a compromise, combining the accessibility of 3-axis machines with the flexibility of additional rotational axes.
The concept of 3+2-axis machining emerged in the late 20th century as manufacturers sought to machine sculptured surfaces—such as turbine blades, molds, and aerospace components—without investing in expensive 5-axis equipment. By adding an indexing table with two discrete rotational axes, 3+2-axis systems allow the workpiece to be reoriented between 3-axis machining operations, effectively accessing multiple surface regions. This approach reduces setup time, improves tool accessibility, and maintains the precision of 3-axis tool paths.
Automated tool path generation for 3+2-axis machining gained traction in the 1990s with advancements in computer-aided design (CAD) and computer-aided manufacturing (CAM) software. Early methods relied on manual surface subdivision and tool path planning, which were time-consuming and prone to errors. The integration of computational geometry, fuzzy clustering, and optimization algorithms in the early 2000s revolutionized the field, enabling automated subdivision of complex surfaces into patches and the generation of optimized tool paths. These developments have made 3+2-axis machining a preferred method for sculptured part production, balancing cost, complexity, and performance.
Fundamentals of 3+2-Axis CNC Machining
Definition and Mechanics
3+2-axis CNC machining, sometimes referred to as positional 5-axis machining, involves a CNC machine with three linear axes (X, Y, Z) and two rotational axes (typically A and B or C). Unlike continuous 5-axis machining, where all axes move simultaneously, 3+2-axis machining fixes the rotational axes at specific angles during each machining operation, effectively reducing the process to a series of 3-axis tool paths. The rotational axes are adjusted between operations to reorient the workpiece, allowing access to different surface regions without repositioning the part manually.
The primary advantage of 3+2-axis machining is its ability to use shorter, more rigid tools, reducing deflection and improving surface finish compared to 3-axis machining alone. Additionally, it simplifies programming and control compared to continuous 5-axis machining, as the tool paths are generated in a 3-axis framework for each fixed orientation.
Sculptured Parts and Their Challenges
Sculptured parts, also known as freeform surfaces, are characterized by complex, non-planar geometries defined by curves and surfaces that lack simple analytical representations. Common examples include turbine blades, automotive body panels, and prosthetic implants. These parts pose several challenges in CNC machining:
-
Geometric Complexity: Sculptured surfaces often have varying curvature, requiring adaptive tool paths to maintain machining accuracy.
-
Tool Accessibility: Concave or undercut regions may be inaccessible in a single setup, necessitating multiple orientations.
-
Surface Finish: Achieving a smooth surface finish requires precise control of scallop height (the residual material between tool passes).
-
Machining Efficiency: Minimizing tool path length and machining time is critical for cost-effectiveness.
Automated surface subdivision and tool path generation address these challenges by breaking down complex surfaces into simpler patches, optimizing tool orientations, and generating efficient tool paths.
Surface Subdivision Techniques
Overview of Surface Subdivision
Surface subdivision is the process of dividing a complex sculptured surface into smaller, simpler patches that can be machined efficiently using 3-axis tool paths. The goal is to create patches with consistent geometric and machinability properties, such as uniform curvature or accessibility, to facilitate tool path generation. Automated surface subdivision reduces manual intervention, improves repeatability, and enhances machining efficiency.
Geometric and Machinability Parameters
The subdivision process begins with the analysis of the surface's geometric and machinability characteristics. Key parameters include:
-
Curvature: Measures the rate of change of the surface's tangent plane, indicating concave, convex, or saddle regions.
-
Surface Normal: Defines the direction perpendicular to the surface at each point, influencing tool orientation.
-
Machinability: Assesses the ease of machining a surface patch based on tool accessibility, cutter size, and material properties.
These parameters are calculated using differential geometry techniques or approximated from discrete surface representations, such as triangular meshes or Non-Uniform Rational B-Splines (NURBS).
Rough Subdivision
The initial step, known as rough subdivision, categorizes the surface into regions based on major shape categories (e.g., convex, concave, or planar). This is typically achieved through:
-
Grid Point Analysis: The surface is represented by a grid of points, and geometric parameters (e.g., curvature, normal vectors) are calculated at each point.
-
Clustering: Points with similar geometric properties are grouped using clustering algorithms, such as k-means or fuzzy pattern clustering, to form preliminary regions.
Rough subdivision ensures that each region has relatively consistent shape characteristics, simplifying subsequent processing.
Fuzzy Pattern Clustering
To optimize the number and boundaries of surface patches, fuzzy pattern clustering techniques are employed. Unlike traditional clustering, fuzzy clustering allows points to belong to multiple clusters with varying degrees of membership, accommodating the gradual transitions in sculptured surfaces. The process involves:
-
Feature Extraction: Geometric and machinability parameters are extracted from grid points.
-
Cluster Optimization: An objective function minimizes the number of patches while ensuring each patch is machinable in a single setup.
-
Patch Number Determination: The algorithm iteratively adjusts the number of clusters to balance complexity and machinability.
Fuzzy clustering enhances the adaptability of subdivision, particularly for surfaces with complex curvature variations.
Boundary Definition with Voronoi Diagrams
After clustering, the boundaries of surface patches are defined to ensure seamless transitions between patches. Voronoi diagrams are used to delineate patch boundaries by:
-
Centroid Identification: Each patch is assigned a characteristic center based on clustered points.
-
Boundary Delineation: Voronoi diagrams partition the surface into regions where each point is closest to a specific centroid, forming precise patch boundaries.
This approach avoids the computational expense of increasing grid density, as it leverages existing points to define boundaries efficiently.
Comparison of Subdivision Techniques
The following table compares common surface subdivision techniques used in 3+2-axis CNC machining:
Technique |
Description |
Advantages |
Limitations |
Applications |
---|---|---|---|---|
Manual Subdivision |
Surface divided manually based on operator expertise |
High control over patch definition |
Time-consuming, error-prone, inconsistent |
Small-scale production, prototyping |
Grid-Based Clustering |
Uses grid points and k-means clustering to group similar regions |
Simple implementation, fast for regular surfaces |
May produce irregular boundaries, less adaptive to complex curvature |
General sculptured surfaces |
Fuzzy Pattern Clustering |
Employs fuzzy logic to allow partial membership in clusters |
Adapts to gradual curvature changes, optimizes patch number |
Computationally intensive, requires parameter tuning |
Complex freeform surfaces |
Voronoi Diagram-Based |
Defines patch boundaries using Voronoi partitioning |
Precise boundary definition, seamless patch transitions |
Dependent on accurate centroid placement, computationally complex |
High-precision parts, molds |
Feature-Based Subdivision |
Divides surface based on geometric features (e.g., edges, curvature extrema) |
Preserves surface features, intuitive for design-driven surfaces |
May produce uneven patches, requires feature detection algorithms |
Aerospace, automotive components |
This table highlights the trade-offs between computational complexity, adaptability, and precision, guiding the selection of subdivision techniques based on surface complexity and application requirements.
Tool Path Generation for 3+2-Axis Machining
Principles of Tool Path Generation
Tool path generation involves creating a sequence of cutter locations (CL) and orientations that guide the tool across each surface patch to remove material while meeting tolerances. In 3+2-axis machining, tool paths are generated as 3-axis paths for each fixed workpiece orientation, with the rotational axes adjusted between patches to optimize accessibility. Key objectives include:
-
Minimizing Tool Path Length: Reduces machining time and energy consumption.
-
Controlling Scallop Height: Ensures surface finish by limiting residual material between passes.
-
Avoiding Interference: Prevents tool collisions with the workpiece or fixtures.
-
Optimizing Tool Orientation: Maximizes machining strip width and efficiency.
Tool Path Strategies
Several strategies are used to generate tool paths for sculptured surfaces in 3+2-axis machining:
-
Iso-Parametric Tool Paths:
-
Follow parametric curves (e.g., u or v directions) on the surface.
-
Simple to implement but may lead cancel redundant passes in regions with low curvature variation.
-
Suitable for surfaces with regular parameterization.
-
-
Iso-Scallop Tool Paths:
-
Maintain constant scallop height by adjusting the path interval based on surface curvature and cutter geometry.
-
Improves efficiency by reducing unnecessary passes in flat regions.
-
Requires complex calculations to evaluate machining strip width.
-
-
Steepest-Directed Tool Paths:
-
Align tool paths along the steepest tangent direction of the surface, mimicking an expert engraver’s approach.
-
Enhances cutting efficiency by matching tool motion to surface geometry.
-
Effective for surfaces with significant curvature gradients.
-
-
Iso-Cusped Tool Paths:
-
Minimize overmachining by ensuring tool paths follow surface contours that prevent redundant material removal.
-
Often combined with steepest-directed paths for optimal results.
-
Automated Tool Path Generation Workflow
The automated tool path generation process for 3+2-axis machining typically follows these steps:
-
Patch Analysis: Each surface patch is analyzed to determine its geometric and machinability properties, including curvature and normal vectors.
-
Orientation Selection: Optimal tool orientations are selected for each patch, typically by aligning the tool axis with the patch’s characteristic normal or maximizing accessibility.
-
Path Planning: 3-axis tool paths are generated for each patch using a chosen strategy (e.g., iso-scallop or steepest-directed).
-
Interference Checking: Algorithms detect potential collisions between the tool, workpiece, and fixtures, adjusting paths or orientations as needed.
-
Path Optimization: Global optimization techniques, such as genetic algorithms, minimize total path length and machining time while maintaining tolerances.
Interference Avoidance
Tool interference, or gouging, occurs when the tool removes unintended material or collides with the workpiece. Common methods to avoid interference include:
-
Cutter Size Selection: Using a cutter with a radius smaller than the smallest concave curvature radius on the surface.
-
Offset Surface Approach: Generating tool paths on an offset surface to avoid contact with critical regions.
-
Interference Detection Algorithms: Analyzing cutter location (CL) data to identify and eliminate interference zones, such as “butterfly” patterns in concave regions.
Comparison of Tool Path Strategies
The following table compares tool path strategies for 3+2-axis CNC machining of sculptured surfaces:
Strategy |
Description |
Advantages |
Limitations |
Applications |
---|---|---|---|---|
Iso-Parametric |
Follows parametric curves on the surface |
Simple, predictable paths |
Inefficient for complex surfaces, may produce uneven scallop heights |
Regular surfaces, prototyping |
Iso-Scallop |
Maintains constant scallop height by adjusting path intervals |
Efficient, uniform surface finish |
Computationally intensive, requires accurate curvature data |
High-precision parts, molds |
Steepest-Directed |
Aligns paths with the steepest tangent direction |
High cutting efficiency, mimics expert engraving |
Complex to compute, may require multiple orientations |
Complex freeform surfaces |
Iso-Cusped |
Minimizes overmachining by following surface contours |
Reduces redundant milling, improves surface quality |
Requires integration with other strategies, computationally complex |
Aerospace, automotive components |
Hybrid (SDIC) |
Combines steepest-directed and iso-cusped paths |
Balances efficiency and quality, shorter paths |
Highly complex, requires advanced optimization algorithms |
High-performance parts, turbine blades |
This table illustrates the trade-offs between simplicity, efficiency, and computational requirements, aiding in the selection of appropriate strategies for specific applications.
Optimization Techniques in Tool Path Generation
Role of Optimization
Optimization is integral to automated tool path generation, as it minimizes machining time, reduces tool wear, and ensures high surface quality. Optimization techniques address multiple objectives, including:
-
Minimizing total tool path length.
-
Reducing machining time by optimizing feed rates and path intervals.
-
Ensuring uniform scallop height for consistent surface finish.
-
Avoiding interference and maintaining tool accessibility.
Genetic Algorithms
Genetic algorithms (GAs) are widely used for multi-objective optimization in tool path generation. GAs mimic natural evolution by:
-
Population Initialization: Creating a set of candidate tool path configurations.
-
Fitness Evaluation: Assessing each configuration based on objectives (e.g., path length, scallop height, interference).
-
Selection and Crossover: Combining high-performing configurations to generate new candidates.
-
Mutation: Introducing random variations to explore new solutions.
-
Iteration: Repeating the process until an optimal solution is found.
GAs are particularly effective for 3+2-axis machining, as they can optimize tool orientations and path intervals simultaneously, balancing conflicting objectives like machining time and surface deviation.
Fuzzy Logic and Neural Networks
Fuzzy logic enhances tool path generation by handling uncertainty in surface geometry and machinability. For example, fuzzy clustering optimizes patch subdivision by allowing points to belong to multiple patches with varying membership degrees. Neural networks, such as Kohonen networks, are used to group surface points based on geometric properties, improving patch smoothness and curvature continuity.
Pareto-Based Optimization
Pareto-based optimization addresses conflicting objectives, such as minimizing machining time while maintaining surface quality. By generating a Pareto front—a set of non-dominated solutions—decision-makers can select the best trade-off based on application requirements. This approach is often combined with genetic algorithms to provide a range of optimal tool path configurations.
Comparison of Optimization Techniques
The following table compares optimization techniques for tool path generation in 3+2-axis CNC machining:
Technique |
Description |
Advantages |
Limitations |
Applications |
---|---|---|---|---|
Genetic Algorithms |
Evolutionary optimization using population-based search |
Handles multi-objective problems, robust |
Computationally intensive, requires parameter tuning |
Complex tool path optimization |
Fuzzy Logic |
Uses fuzzy sets to handle uncertainty in geometry and machinability |
Adapts to complex surfaces, improves clustering |
Complex to implement, may require expert knowledge |
Surface subdivision, patch optimization |
Neural Networks |
Groups surface points based on learned geometric patterns |
Improves patch smoothness, handles non-linearities |
Requires training data, computationally expensive |
Surface feature recognition |
Pareto-Based Optimization |
Generates trade-off solutions for conflicting objectives |
Provides flexibility in decision-making |
Requires post-processing to select final solution |
Multi-objective tool path planning |
Simulated Annealing |
Iterative optimization inspired by material cooling |
Simple to implement, effective for local optimization |
May converge slowly, less effective for multi-objective problems |
Path interval optimization |
This table highlights the strengths and weaknesses of each technique, guiding their application in automated tool path generation.
Implementation and Software Tools
CAD/CAM Integration
Automated surface subdivision and tool path generation are typically implemented within CAD/CAM software, which integrates surface modeling, tool path planning, and CNC code generation. Common software platforms include:
-
Siemens NX: Offers advanced surface subdivision and tool path generation for 3+2-axis machining.
-
Autodesk PowerMill: Supports automated tool path optimization and interference checking.
-
Mastercam: Provides flexible tool path strategies for sculptured surfaces.
-
CATIA: Widely used in aerospace for complex surface machining.
These platforms leverage computational geometry libraries, such as ACIS, to model surfaces and generate tool paths.
Programming and Simulation
Tool paths are programmed using G-code, which specifies cutter locations, feed rates, and spindle speeds. Simulation tools within CAM software visualize tool paths, detect collisions, and estimate machining time. Advanced systems use digital twins—virtual replicas of the CNC machine and workpiece—to validate tool paths before machining.
Case Study: Turbine Blade Machining
Consider a turbine blade with a complex freeform surface, requiring high precision and smooth surface finish. The surface is subdivided into convex and concave patches using fuzzy clustering and Voronoi diagrams. For each patch, iso-scallop tool paths are generated with a 3 mm ball-end mill, maintaining a scallop height of 0.01 mm. Tool orientations are optimized to minimize path length, and interference is avoided using offset surface techniques. The resulting tool paths reduce machining time by 20% compared to manual methods, demonstrating the efficacy of automation.
Applications of 3+2-Axis CNC Machining
Aerospace Industry
In aerospace, 3+2-axis machining is used to produce turbine blades, airfoils, and structural components with complex geometries. Automated tool path generation ensures tight tolerances and smooth surfaces, critical for aerodynamic performance.
Automotive Industry
Automotive manufacturers employ 3+2-axis machining for molds, dies, and body panels. The ability to machine complex surfaces in multiple orientations reduces production time and enhances design flexibility.
Medical Device Manufacturing
Sculptured surfaces in medical implants, such as hip joints and dental prosthetics, require high precision and biocompatibility. 3+2-axis machining with automated tool paths ensures accurate geometry and smooth finishes, improving patient outcomes.
Mold and Die Making
Mold and die production benefits from 3+2-axis machining’s ability to handle intricate cavities and undercuts. Automated subdivision and tool path generation streamline the process, reducing lead times and costs.
Challenges and Limitations
Despite its advantages, automated surface subdivision and tool path generation for 3+2-axis machining face several challenges:
-
Computational Complexity: Algorithms like fuzzy clustering and genetic optimization require significant computational resources, limiting real-time applications.
-
Surface Representation: Discrete representations (e.g., triangular meshes) may introduce errors in curvature calculations, affecting patch quality.
-
Tool Path Smoothness: Transitions between patches or orientations can cause discontinuities, impacting surface finish.
-
Scalability: Handling large, highly complex surfaces may overwhelm current algorithms, requiring advanced hardware or simplified models.
Future Research Directions
The field of automated surface subdivision and tool path generation is poised for significant advancements. Key research directions include:
-
Machine Learning Integration: Leveraging deep learning to predict optimal patch divisions and tool paths based on historical machining data.
-
Real-Time Optimization: Developing algorithms for on-the-fly tool path adjustment during machining, enhancing adaptability.
-
Hybrid Machining Strategies: Combining 3+2-axis and continuous 5-axis techniques to balance cost and complexity.
-
Sustainability: Optimizing tool paths to minimize energy consumption and material waste, aligning with green manufacturing goals.
Conclusion
Automated surface subdivision and tool path generation for 3+2-axis CNC machining of sculptured parts represent a transformative approach to modern manufacturing. By dividing complex surfaces into manageable patches and generating optimized tool paths, these techniques enhance efficiency, precision, and scalability across industries. Advances in computational geometry, optimization algorithms, and CAD/CAM integration have made automation a cornerstone of 3+2-axis machining, with applications ranging from aerospace to medical devices. While challenges remain, ongoing research in machine learning, real-time optimization, and sustainable manufacturing promises to further elevate the capabilities of this technology, solidifying its role in the future of precision engineering.
Reprint Statement: If there are no special instructions, all articles on this site are original. Please indicate the source for reprinting:https://www.cncmachiningptj.com/,thanks!
3, 4 and 5-axis precision CNC machining services for aluminum machining, beryllium, carbon steel, magnesium, titanium machining, Inconel, platinum, superalloy, acetal, polycarbonate, fiberglass, graphite and wood. Capable of machining parts up to 98 in. turning dia. and +/-0.001 in. straightness tolerance. Processes include milling, turning, drilling, boring, threading, tapping, forming, knurling, counterboring, countersinking, reaming and laser cutting. Secondary services such as assembly, centerless grinding, heat treating, plating and welding. Prototype and low to high volume production offered with maximum 50,000 units. Suitable for fluid power, pneumatics, hydraulics and valve applications. Serves the aerospace, aircraft, military, medical and defense industries.PTJ will strategize with you to provide the most cost-effective services to help you reach your target,Welcome to Contact us ( sales@pintejin.com ) directly for your new project.
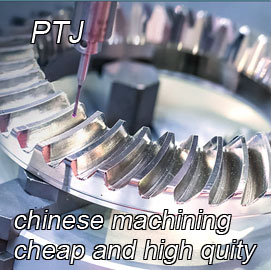
- 5 Axis Machining
- Cnc Milling
- Cnc Turning
- Machining Industries
- Machining Process
- Surface Treatment
- Metal Machining
- Plastic Machining
- Powder Metallurgy Mold
- Die Casting
- Parts Gallery
- Auto Metal Parts
- Machinery Parts
- LED Heatsink
- Building Parts
- Mobile Parts
- Medical Parts
- Electronic Parts
- Tailored Machining
- Bicycle Parts
- Aluminum Machining
- Titanium Machining
- Stainless Steel Machining
- Copper Machining
- Brass Machining
- Super Alloy Machining
- Peek Machining
- UHMW Machining
- Unilate Machining
- PA6 Machining
- PPS Machining
- Teflon Machining
- Inconel Machining
- Tool Steel Machining
- More Material